Stinson 108-3
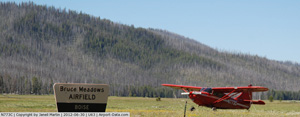
Photo copyright Jenell Martin - used with permission
Private Pilot, N773C
Stanley, Idaho
June 30, 2012
On June 30, 2012, a pilot with three adult male passengers flew a 1947 Stinson 108-3, N773C, into Bruce Meadows Airport (U63) in Stanley, Idaho. Bruce Meadows Airport has a 5,000-foot turf-dirt runway at an elevation of 6,370 feet mean sea level. Prior to departure, the pilot determined the temperature to be 84°F, the density altitude to be around 9,200 feet, and calculated the weight of the plane to be 2,314 pounds, or only 86 pounds less than the aircraft’s maximum gross weight of 2,400 pounds.
At about 1415, the pilot with his passengers began to takeoff from Runway 23, with wind from 30° at 10 knots, gusting to 20 knots, which made for almost a direct tailwind. The aircraft accelerated down the runway but still had not become airborne after using three quarters of the nearly mile-long runway. The pilot stated he was about to abort the takeoff due to excessive ground roll, when a gust of wind lifted the airplane into the air. However, when the airplane failed to climb, he attempted to locate a suitable field to perform a forced landing. Prior to executing the forced landing, the pilot said the airplane encountered a downdraft and subsequently impacted a stand of trees. He sustained serious injuries, while the three passengers received only minor injuries. No mechanical malfunctions were discovered that would have precluded normal operations of the airplane or engine during the post-accident examination.
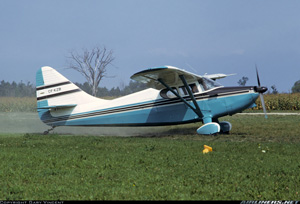
Photo copyright Gary Vincent - used with permission
History of Flight
The flight to Bruce Meadows Airport (U63) was part of a hiking trip for three passengers. Bruce Meadows Airport is one of 26 active public use remote runways located in the Idaho wilderness of the Frank Church-River of No Return Wilderness Area. The U.S. Department of Agriculture's Forestry Service describes the Frank Church-River of No Return as a wilderness of steep, rugged mountains, deep canyons, and wild, whitewater rivers. There are warnings to pilots that there are a number of smaller landing strips, and they are extremely demanding of the highest degree of mountain flying skills. One of these warnings includes the recommendation that pilots familiar with canyon and short-field operation should only attempt these landing strips.
Bruce Meadows Airport has a 5,000-foot by 110-foot turf-dirt runway at an elevation of 6,370 feet mean sea level located 20 miles northwest of Stanley, Idaho. The airport information provides the following remarks: "Normally land Runway 05, depart Runway 23, when wind conditions permit. Airport is located in a high mountain valley surrounded by high terrain. Very high density altitudes in summer."
The flight into Bruce Meadows Airport was uneventful and the group arrived that morning onboard a 1947 Stinson 108-3 Voyager that was owned and piloted by one member of the group.
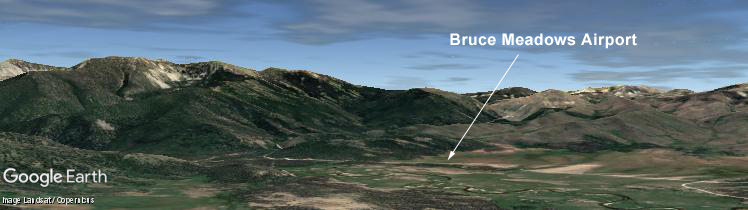
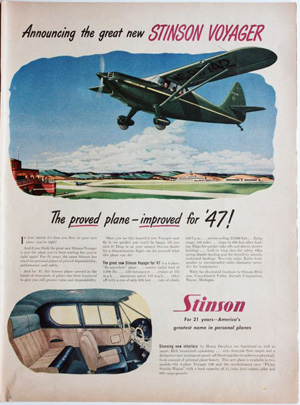
View Larger
Stinson 108-3
The Stinson 108-3 is a four seat, tube and fabric high-wing design with conventional landing gear, typical of aircraft built in the 1945-1950s. There were over 5,260 aircraft produced with earlier variants being powered by 150 HP engine. Later models, like the accident aircraft, were powered by the 165 HP Franklin engine. The Stinson 108 is known for its docile flight characteristics and the landing gear could be fitted with wheels, floats or skis. There was one model with a wood paneling interior described as the "Flying Station Wagon." Partial leading edge slots located on the outboard portion of the wing allow the ailerons to remain unstalled at high angles of attack, and an adequate amount of useful load makes the Stinson 108 a commonly used aircraft for backcountry or bush flying.
Investigators learned that after spending the morning hiking trails, the men decided to fly to McCall Municipal Airport (MYL) in McCall, Idaho for dinner. Not unfamiliar with high density altitude operations, the pilot calculated his aircraft takeoff performance for what was an 84°F day.
Investigators established the pilot calculated the density altitude to be 9,200 feet. The pilot had made four previous flights to Bruce Meadows Airport, two of which were in the summer months, none with four people onboard or at max gross weight. The Stinson 108-3 has a total fuel capacity of 50 gallons with fuel tanks incorporated into each wing. The pilot was aware there were 20 gallons of fuel on board for takeoff. When the pilot computed the takeoff weight of the Stinson with all four people aboard, he determined the weight to be 2,314 pounds, 86 pounds below the maximum gross weight of 2,400 pounds.
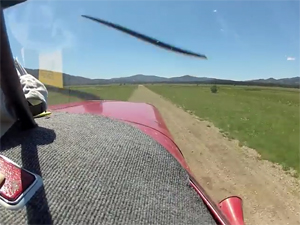
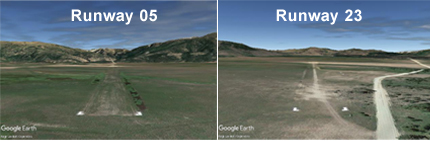
View Larger
At 1415, the pilot taxied the Stinson to the end of Runway 23 and noted that the winds were 30° at 10 knots gusting to 20 knots. The pilot elected to take off with an almost direct tailwind because the terrain rises rapidly to the northeast from Runway 05, whereas Runway 23 has a more gradual rise in terrain. The pilot applied full power and the aircraft began to accelerate down the 5,000 foot turf-dirt runway. The plane was on the ground three-quarters of the way down the runway. Due to the excessive ground roll, the pilot prepared to abort the takeoff just before a gust of wind lifted the plane into the air.
The airplane, with the wings near stall, at maximum gross weight, and with the engine producing less than its 165 HP capability, the Stinson was unable to climb. With the plane not gaining altitude, the pilot attempted to locate an open field to perform a forced landing. However, the aircraft encountered a downdraft, causing the aircraft to collide with a stand of trees, approximately three miles from the beginning of takeoff. The airplane was substantially damaged and the pilot sustained serious injuries. The three passengers received minor injuries. Nearby campers and first responders were able to provide assistance.

View Larger
One of the passengers recorded the entire takeoff-to-crash sequence on a video camera.
Aircraft and Performance Charts
The NTSB cited that the pilot's decision to operate the aircraft outside of its performance envelope was one of the causal factors in the crash. In 1947, the Stinson 108 was certified by the Civil Aeronautics Administration (CAA), which was the predecessor to today's Federal Aviation Administration (FAA). The certification requirements for aircraft performance at the time could be found in the Civil Air Regulations, Part 3 (CAR-3), but they were much less descriptive than what is required by today's Title 14 Code of Federal Regulations (CFR), Part 23. When the aircraft was certificated, there also was no standard of what had to be included in the Aircraft Flight Manual (AFM) or Pilot Operating Handbook (POH). It would not be until 1976, when the General Aviation Manufacturing Association (GAMA) developed the standardized POH requirements that are used today. For example, the carburetor fuel leaning procedure is only found in the Franklin engine manual.
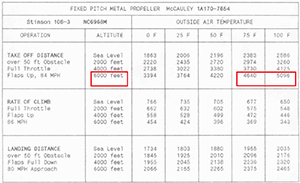
View Larger
In 1947, each Stinson 108 had its own individualized five-page AFM because the equipment list and the weight and balance pages specific to that aircraft were included in the manual. On page five of the Stinson's AFM, the pilot found the Takeoff and Landing Performance Chart for Fixed Pitch Metal Propellers. The chart contained takeoff distance, rate of climb, and landing distance for sea level at 2,000, 4,000, and 6,000 feet. The temperatures listed were from 0°F to 100°F in 25°F increments. Without taking into consideration the temperature, the elevation of the airport was already 370 feet higher than the highest altitude listed in the performance charts. Based on the information available, the pilot should not have attempted a takeoff.
The calculated performance for the airplane exceeds the available takeoff field length with both ground run and over a 50-foot obstacle. It should also be noted that the performance numbers in the AFM are the best performance the airplane can deliver.
The effects of time and age as well as pilot skill level were not accounted for in the original establishment of the Takeoff and Landing Performance Chart for this Stinson. When the chart was generated, the aircraft was brand new, had a new engine, and was probably flown by a proficient test pilot under ideal conditions. On the day of the accident, the aircraft had approximately 1,550 hours on the aircraft, the engine had 230 hours since overhaul, the pilot was experienced, and the takeoff was going to be from a turf-dirt strip with a tailwind. Realizing that most pilots and older aircraft will not match the published performance figures has led to the practice by many pilots to add a 30%-50% safety factor to the number they calculate to ensure they will have enough runway to takeoff or land on. Pilots should pay attention to the notes in the performance charts for items such as flap setting or head wind, zero wind or tailwind speeds used when generating the chart. This information is vital to achieving the book values. For new aircraft, the requirement states that the procedures used for determining takeoff and landing distances must be executable consistently by pilots of average skill in atmospheric conditions expected to be encountered in service.
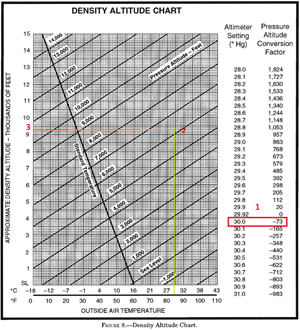
View Larger
Density Altitude
Density altitude has significant effects on aircraft performance such as the lift generated by the wings, the efficiency of the propeller, and the power output of the engine. These effects cause the aircraft to accelerate slower on takeoff, require a longer takeoff to achieve a higher true airspeed in order to attain the same amount of lift, and to have a lower rate of climb as a result of reduced lift and power generation. Most pilots are aware of these effects, but unfortunately most pilots do not fully grasp the real concept of density altitude until they experience it in the cockpit of an underperforming aircraft. Effects of density altitude can be demonstrated, conceptualized, or felt, but many pilots would have a difficult job of describing the phenomenon to another person. Even the simple definition of density altitude, that it is the pressure altitude adjusted for a non-standard temperature, provides little clarity of what is really going on.
Since all aircraft performance is compared and evaluated using the standard atmosphere, all aircraft instruments are calibrated for the standard atmosphere. Thus, certain corrections must apply to the instrumentation, as well as the aircraft performance, if the actual operating conditions do not fit the standard atmosphere. In order to account properly for the nonstandard atmosphere, certain related terms must be defined.
As stated in the Pilots Handbook of Aeronautical Knowledge, "the pressure of the atmosphere may vary with time, but, more importantly, it varies with altitude and temperature. Due to the changing atmospheric pressure, a standard reference was developed. The standard atmosphere at sea level has a surface temperature of 59°F or 15 degrees Celsius (°C) and a surface pressure of 29.92 inches of mercury ("Hg) or 1013.2 millibars (mb)."
"Pressure altitude is the height above the standard datum plane. The standard datum plane is a theoretical level at which the pressure of the atmosphere is 29.92 "Hg and the weight of air is 14.7 psi. As atmospheric pressure changes, the standard datum plane may be below, at, or above sea level. If the altimeter is set for 29.92 "Hg, the altitude indicated is the pressure altitude or rather is it is the altitude in the standard atmosphere corresponding to the sensed pressure."
"Density altitude is pressure altitude corrected for nonstandard temperature. As the density of the air increases (lower density altitude), aircraft performance increases. Conversely, as air density decreases (higher density altitude), aircraft performance decreases. A decrease in air density means a high density altitude; an increase in air density means a lower density altitude."
"Density altitude is determined by first finding pressure altitude and then correcting this altitude for nonstandard temperature variations. Using a flight computer, density altitude can be computed by inputting the pressure altitude and outside air temperature at flight level. Density altitude can also be determined by referring to density altitude tables and charts."
"Air density is affected by changes in altitude, temperature, and humidity. High density altitude refers to thin air while low density altitude refers to dense air. The conditions that result in a high density altitude are high elevations, low atmospheric pressures, high temperatures, high humidity, or some combination of these factors. Lower elevations, high atmospheric pressure, low temperatures, and low humidity are more indicative of low density altitude. Regardless of the actual altitude at which the aircraft is operating, it will perform as though it were operating at an altitude equal to the existing density altitude. Density altitude can be thought of as the vertical distance above sea level in the standard atmosphere at which a given density is to be found. Since aircraft performance data at any level is based upon air density under standard day conditions, such performance data applies to air density levels that may not be identical to altimeter indications. Under conditions higher or lower than standard, these levels cannot be determined directly from the altimeter."
The FAA and many other aviation organizations provide many sources of useful information for preflight planning, through books, articles, or videos. These sources also provide training on specific types of flying such as mountain flying and the FAA advocates getting professional training from a Certified Flight Instructor familiar with the specific type of operation before flight.
View: Off Airport Guide
View: FAA Safety Team Mountain Flying
View: The Zen of Mountain Flying
In order to understand how air density affects aircraft performance, one needs to understand its relationship to the generation of lift. An explanation of lift can be traced back to Sir Isaac Newton's Laws of Motion and the work of Daniel Bernoulli, a Swiss mathematician, who determined that the pressure of a moving fluid (liquid or gas) is inversely proportional to the velocity squared. This principle is used to explain what happens to air passing over the curved top of the airplane wing.
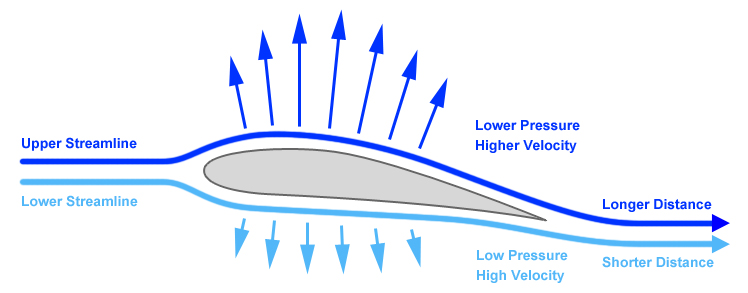
Since air is a fluid, as the wing moves through the air, the flow of air hits the leading edge of the wing where it splits, and is forced to travel either over the top or bottom of the wing. As a result of the airfoil's camber, air must travel a further distance across the top of the wing than for the bottom of the wing. In order for the air over the top of the wing to travel a further distance yet arrive at the same point as air moving a shorter distance under the wing, the air over the top of the wing must travel at a higher velocity than the bottom. This higher velocity air moving over the curved top surface creates a low-pressure area that draws the wing upward, and is the principle of lift. The lift equation is used to analyze this relationship between velocity and lift generation.
L = (CL·ρ·V2·S)/2
Where lift (L) is determined through the relationship of the air density (ρ), the airfoil velocity (V), the surface area of the wing (S) and the coefficient of lift (CL) for a given airfoil.
When considering a basic wing airfoil without the use of lift generating devices such as flaps, it can be assumed that the coefficient of lift (CL) at a given angle of attack (AOA) and the area of the wing (S) are constant or do not change. The only variables that do change are the velocity (V) and density(ρ). Lift is proportional to the square of the aircraft's velocity. For example, an airplane traveling at 200 knots has four times the lift as the same airplane traveling at 100 knots if the AOA and other factors remain constant. Since the velocity term is squared, changes in velocity affect the amount of lift generated faster than the variation in density.
In order to produce the same amount of lift when the density changes, a higher velocity would be required for a high density altitude, and a lower velocity would be required for a low density altitude. This is why aircraft performance seems to increase in the winter, due to the thick dense air associated with low density altitude, and decrease during the summer, due to the thin air from a high density altitude.
The total lift must equal the total weight of the airplane to remain in flight, so if the density is decreased (low density), it follows to reason that one of the other factors must be increased. The factors usually increased are airspeed or AOA of the wing because these are controlled directly by the pilot. For example, when the airspeed is low, the AOA (coefficient of lift) must be relatively high if the balance between lift and weight is to be maintained. If thrust decreases and airspeed decreases, lift becomes less than weight and the aircraft starts to descend. To maintain level flight at a given airspeed, the pilot adjusts the AOA to an amount that generates a lift force equal to the weight of the aircraft. The AOA is adjusted to maintain lift equal weight. However, a pilot can only increase the AOA to a point known as the critical AOA where the airstream flowing over the top surface of the wing separates and the wing becomes stalled. The critical AOA is also where the coefficient of lift is at its maximum value. Once a wing is at the critical AOA, it cannot increased more, and increasing the velocity is the only variable that continues to manipulate the amount of lift generated. Just like the other variable, there is a limit to the maximum velocity an airplane can achieve based on engine power and structural limits. When the velocity and coefficient of lift are at their maximum and they still cannot generate the amount of lift that is required, the pilot may either wait for a cooler temperature or decrease the weight of the aircraft.
Lift and drag vary directly with the density of the air. At an altitude of 18,000 feet, the density of the air has one-half the density of air at sea level.
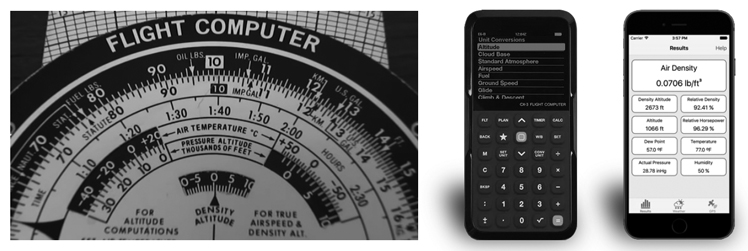
Due the ease of access to information, cellphones, tablet computers, and flight applications have changed the way pilots operate. There are a variety of approved aviation applications that provide information such as: weight and balance calculations, takeoff and landing data, crosswind component, electronic E6B functions, weather, airport information, fuel prices, Notice to Airmen (NOTAMs) and Temporary Flight Restrictions (TFRs). These tools offer a pilot the opportunity to take advantage of new technology.
Carburetor and Mixture
Investigators also noted that the pilot took off with the engine in a full-rich position. The Franklin engine operating instructions provided leaning procedures at altitudes greater than 3,000 feet to maximize engine RPM.
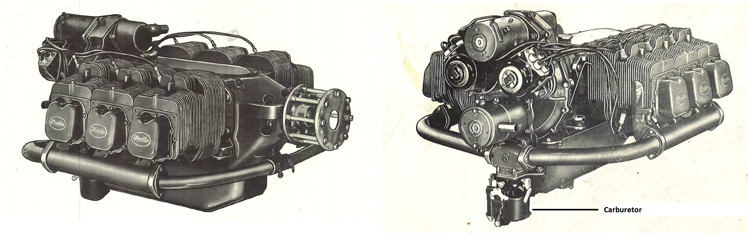
The lower density of air available at altitude also affects the amount of horsepower an engine can produce. The rule of thumb is that a normally aspirated engine loses 3-4% horsepower for every 1,000 feet above sea level. The Stinson 108 had a 165 HP engine, and although the airplane was sitting at 6,370 feet, due to the density altitude, the volume of air available would be equivalent to the volume of air at 9,000 feet on a standard day. Applying the rule of thumb, this would mean that the Stinson was going to produce 27-36% less power, or that the engine would only produce 129-138 HP at full power, assuming the proper air/fuel ratio is used. Since the pilot left the mixture in the full rich position, the air/fuel ratio would have been overly rich or more than optimal, causing the engine to produce even less horsepower.
Conclusion
Investigators determined that the pilot failed to account for the detrimental effects of high density altitude. While he properly assessed the density altitude, he did not apply it correctly to his airplane and elected to takeoff despite being substantially outside the aircraft's approved performance capability.
The National Transportation Safety Board (NTSB) determined the probable cause of this accident to be the pilot's inadequate preflight planning and decision to takeoff at a density altitude outside of the airplane's takeoff performance envelope, with a tailwind, and near the airplane's maximum gross weight, which resulted in the airplanes inability to climb and clear trees.
The complete text of the accident report is available at the following link: Accident Report
The NTSB cited inadequate preflight planning as the probable cause of this accident. Title 14 Code of Federal Registration (CFR) 91.103 covers what the FAA requires for preflight planning.
§91.103 Preflight action.
Each pilot in command shall, before beginning a flight, become familiar with all available information concerning that flight. This information must include:
- For a flight under IFR or a flight not in the vicinity of an airport, weather reports and forecasts, fuel requirements, alternatives available if the planned flight cannot be completed, and any known traffic delays of which the pilot in command has been advised by ATC.
- For any flight, runway lengths at airports of intended use, and the following takeoff and landing distance information
- For civil aircraft for which an approved Airplane or Rotorcraft Flight Manual containing takeoff and landing distance data is required, the takeoff and landing distance data contained therein; and
- For civil aircraft other than those specified in paragraph (b)(1) of this section, other reliable information appropriate to the aircraft, relating to aircraft performance under expected values of airport elevation and runway slope, aircraft gross weight, and wind and temperature.
The Pilot's Handbook of Aeronautical Knowledge (approximately 58MB), has an entire chapter dedicated to Aeronautical Decision-Making and Advisory Circular 60-22 addresses the issue as well. The following is an excerpt from that chapter, which provides pilots with a tool to safely identify and mitigate risk of the upcoming flight, during preflight planning.
AC 60-22 Aeronautical Decision Making Chapters 1-3
AC 60-22 Aeronautical Decision Making Chapter 4-Appendix
The PAVE Checklist
By incorporating the PAVE checklist into preflight planning, the pilot divides the risks of flight into four categories: Pilot-in-command (PIC), Aircraft, enVironment, and External pressures (PAVE) which form part of a pilot's decision-making process.
View PAVE
For determining aircraft performance requirements, the certification basis was 1947 Civil Air Regulations, Part 3 Type Certificate 767. These are the rules applicable to the certification basis of the Stinson 108.
1947 Civil Air Regulations, Part 3 (CAR-3) Takeoff Performance Data Requirements
§ 3.84 Take-off.
- The distance required to take off and climb over a 50-foot obstacle shall be determined under the following conditions:
- Most unfavorable combination of weight and center of gravity location,
- Engines operating within the approved limitations,
- Cowl flaps in the position normally used for take-off.
- Upon obtaining a height of 50 feet above the level take-off surface, the airplane shall have attained a speed of not less than 1.3 Vs1 unless a lower speed of not less than Vx plus 5 can be shown to be safe under all conditions, including turbulence and complete engine failure.
- The distance so obtained, the type of surface from which made, and the pertinent information with respect to the cowl flap position, the use of flight-path control devices and landing gear retraction system shall be entered in the Airplane Flight Manual. The take-off shall be made in such a manner that its reproduction shall not require an exceptional degree of skill on the part of the pilot or exceptionally favorable conditions.
Performance Data
In 1947 the CAR 3 regulations did not specify a max altitude or temperature range to be used in the performance chart. Manufacturers would pick a range of normal conditions for which their aircraft might operate, submit the performance data to the CAA, and, if approved, ensure the aircraft meets the requirements.
The current Airworthiness Standards for Normal Category Aircraft under 14 CFR Part 23, as amended, went into effect on August 15, 2017. Part 23 describes the requirements and information that manufacturers of aircraft must supply to the FAA and/or owner or operator. The performance charts require airport elevations from sea level to 10,000 feet and temperatures above and below standard day temperature that are within the range of operating limitations.
Although the performance requirements have changed since 1947 when the Stinson 108 was produced, their intent has remained the same.
Current Code of Federal Regulations, Part 23 Takeoff Performance Data Requirements
Subpart B-Flight
PERFORMANCE
Airplane certification levels are:
-
Level 1-for airplanes with a maximum seating configuration of 0 to 1 passengers.
-
Level 2-for airplanes with a maximum seating configuration of 2 to 6 passengers.
-
Level 3-for airplanes with a maximum seating configuration of 7 to 9 passengers.
-
Level 4-for airplanes with a maximum seating configuration of 10 to 19 passengers.
Airplane performance levels are:
-
Low speed-for airplanes with a VNO and VMO ≤ 250 Knots Calibrated Airspeed (KCAS) and a MMO ≤ 0.6.
-
High speed-for airplanes with a VNO or VMO > 250 KCAS or a MMO > 0.6.
§23.2105 Performance data.
- Unless otherwise prescribed, an airplane must meet the performance requirements of this subpart in-
- Still air and standard atmospheric conditions at sea level for all airplanes; and
- Ambient atmospheric conditions within the operating envelope for levels 1 and 2 high-speed and levels 3 and 4 airplanes.
- Unless otherwise prescribed, the applicant must develop the performance data required by this subpart for the following conditions:
- Airport altitudes from sea level to 10,000 feet (3,048 meters); and
- Temperatures above and below standard day temperature that are within the range of operating limitations, if those temperatures could have a negative effect on performance.
- The procedures used for determining takeoff and landing distances must be executable consistently by pilots of average skill in atmospheric conditions expected to be encountered in service.
- Performance data determined in accordance with paragraph (b) of this section must account for losses due to atmospheric conditions, cooling needs, and other demands on power sources.
§23.2115 Takeoff performance.
- The applicant must determine airplane takeoff performance accounting for-
- Stall speed safety margins.
- Minimum control speeds; and
- Climb gradients.
- For single engine airplanes and levels 1, 2, and 3 low-speed multiengine airplanes, takeoff performance includes the determination of ground roll and initial climb distance to 50 feet (15 meters) above the takeoff surface.
- For levels 1, 2, and 3 high-speed multiengine airplanes, and level 4 multiengine airplanes, takeoff performance includes a determination the following distances after a sudden critical loss of thrust-
- An aborted takeoff at critical speed.
Ground roll and initial climb to 35 feet (11 meters) above the takeoff surface; and net takeoff flight path.
This flight was conducted under 14 CFR Part 91. The accident report makes no mention of any prevailing cultural or organizational factors that may have caused or prevented this accident.
- The pilot failed to account for the detrimental effects of high-density altitude
- This takeoff was outside the performance capability of the aircraft and should not have been attempted
- The pilot failed to abort the takeoff attempt even though it appeared the plane was not going to get airborne
Pilots will review performance charts for each departure and destination airport per 14 CFR § 91.103.
- The pilot attempted a take-off without proper review of take-off performance data, and therefore did not evaluate environmental conditions, airport elevation, runway length and slope, aircraft gross weight, and wind and temperature.
Pilots will not attempt to extrapolate data from takeoff performance charts when data is non-existent.
- The NTSB concluded that during his preflight planning the pilot should have identified that his aircraft would not have the performance required, given its planned weight, density altitude, and other factors, and he should not have attempted to takeoff.
Airplane Life Cycle
- Operational
Accident Threats
- Loss of Control
Operations
- Personal
Accident Common Themes
- Human Error
- Flawed Assumptions
Human Error
The NTSB concluded that, during his preflight planning, the pilot should have identified that his aircraft would not have the performance required given its planned weight, density altitude, and other factors, and he should not have attempted to takeoff.
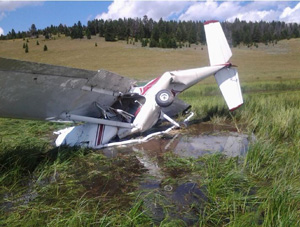
Photo copyright NTSB - used with permission
The NTSB database has many examples of density altitude related accidents. Due to continuing density related accidents, there is a need for further education in density altitude and its effects on aircraft performance. The following accidents are similar to this Stinson 108 accident:
NTSB Identification: WP14LA305
Aircraft: Cessna 150M, registration: N66255
Accident Occurred: July 21, 2014, in Russian Flat, MT
Airport Elevation: 6,336 MSL
Injuries: 1 Fatal
The commercial pilot attempted to take off from a grass runway with an uphill grade and the airplane impacted the terrain about 570 feet beyond the end of the runway. Post-accident examination revealed no mechanical malfunctions that would have precluded normal operation.
The density altitude at the time of the accident was calculated to be over 8,200 feet. Review of airplane manufacturer's takeoff performance charts revealed that the conditions present at the time of the accident exceeded the airplane's takeoff performance limitations.
Probable Cause
The NTSB determined the probable cause of this accident to be the pilot's inadequate preflight performance planning and his operation of the airplane outside of the manufacturer's specified performance limitations.
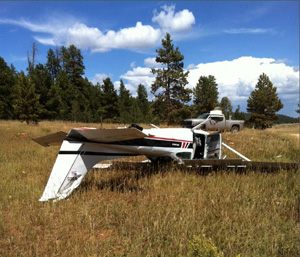
Photo copyright NTSB - used with permission
NTSB Identification: WP11LA400
Aircraft: Cessna 182Q, registration: N79ES
Accident Occurred: August 21, 2011, in Alton, UT
Airport Elevation: 7,780 MSL
Injuries: 3 none
The airplane was loaded about 10 percent below its maximum gross weight, a high-density altitude (10,700 feet) and gusting crosswind conditions existed, when the pilot began the takeoff. The pilot initiated rotation about the time he reached the end of the runway, and the airplane lifted off the ground but did not gain sufficient altitude to avoid striking a fence. During the post-accident examination, no mechanical failures or malfunctions were identified that would have precluded normal operation. According to the airplane's information manual, at the reported weight and weather conditions, the airplane should have been able to take off after traveling less than 1/2 of the distance down the runway. Therefore, the pilot should have had ample time to determine that the airplane was not performing as expected and to abort the takeoff.
Probable Cause
The NTSB determined the probable cause of this accident to be the pilot's failure to abort the takeoff in a timely manner, resulting in insufficient altitude to clear obstructions.
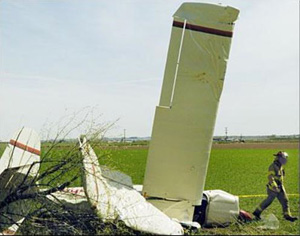
Photo copyright NTSB - used with permission
NTSB Identification: DEN05CA073
Aircraft: Champion 7ECA, registration: N11065
Accident Occurred: June 10, 2005, in Ft. Collins, CO
Airport Elevation: 5,016 MSL
Injuries: 2 Minor
The pilot stated the airplane was loaded "right at gross weight." He stated that approximately 200 feet agl and 3/4 down the runway, the airplane "stopped climbing and started sinking." The pilot attempted to increase the airspeed by lowering the nose; however, the airplane continued to descend. The pilot reduced the power to avoid the power lines and the airplane impacted a tree. An FAA inspector stated the airplane was overloaded and exceeded the gross takeoff weight by at least 18 pounds. Calculated density altitude was 6,500 feet.
Probable Cause
The NTSB determined the probable cause of this accident to be the pilot's inadequate preflight planning/preparation which failed to assure adequate takeoff performance to clear obstacles.
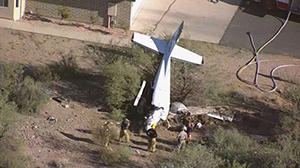
Photo copyright KPHO/KTVK - used with permission
NTSB Identification: WPR16FA176
Aircraft: Cessna 310N, registration: N126P
Accident Occurred: September 9, 2016
Airport Elevation: 2,378 MSL
Injuries: 4 Serious
The airline transport pilot and three passengers were departing in the multi-engine airplane when, during the takeoff, or shortly after rotation, the right engine experienced a total loss of power. The power loss occurred at a time when the airplane was close to or just below the manufacturer's recommended "safe single-engine speed." The hilly terrain surrounding the airport left the pilot with few options for a safe climb out. Further hindering the takeoff was the airplane's anemic single-engine climb performance due to the high-density altitude conditions (4,200 feet) and the airplane's weight. As a result, shortly after the loss of power, the airplane collided with the ground and sustained substantial damage.
The pilot and passengers all sustained serious injuries.
Examination of the right engine revealed that a clear, gelatinous substance had blocked the inlet port of the fuel flow transducer, leading to fuel starvation. The specific source of contamination could not be determined.
Probable Cause
The NTSB determined the probable cause of this accident to be total loss of power to the right engine during takeoff due to a fuel contaminant, which blocked the fuel flow transducer and resulted in fuel starvation to the engine.
Technical Related Lesson
Increased density altitude decreases both engine and airplane performance. Pilots must understand this influence and account for these effects. (Threat Category: Loss of Control)
- The following conditions lower the density of the air and increase density altitude while reducing airplane performance high altitude, low pressure, high temperature, and high humidity.
Common Theme Related Lesson
To ensure safe aircraft take-off and performance operations, a pilot must take into consideration all factors that impact air density. (Common Theme: Human Error)
- Attempting a take-off without proper review of take-off performance data can lead to human error aircraft accidents. By evaluating environmental conditions such as airport elevation, runway length and slope, aircraft gross weight; and wind and temperature, a pilot can determine if a departure can be successful.